
In the
first part, we talked about small temperature fluctuations in the cosmic microwave background radiation (CMI). Now we will switch to another component of the CMBI, approximately 100 times smaller than the temperature signal: polarization. And although we are discussing concepts that are loosely connected with our daily experience, it is necessary to remember that the residual radiation of the Big Bang is, in fact, just light. And light is an electromagnetic wave, an oscillating set of electric fields (E) and magnetic fields (B) propagating at the speed of light.

We immediately say that since photons have electric and magnetic fields that differ from each other, but are related, the presence of polarization can manifest itself in the form of an
E-mode (irrotational component) and a
B-mode (vortex). The recent excitement associated with the prehistoric B-modes in the polarization of the CMBR, and the possibility of their direct detection deserves to be known. They will give us the easiest access to information about the energy involved during inflation, one of the earliest stages in the development of the Universe, whose traces can be found in some other measurable parameters. B-mods are only part of the entire polarization history.
')
Light KMFI - not just a glow
In a nutshell, we recall the first part: the largest signal of the CMBR is present in the form of temperature fluctuations of light (or photons). A sea of ββfree electrons and photons interact with each other very often (through Thomson scattering), and the electrons remain free, since photons have enough energy to keep electrons from combining with atomic nuclei. Electrons are associated with photons, and at the same time they are constantly moving between regions of high density formed by clumping dark matter.
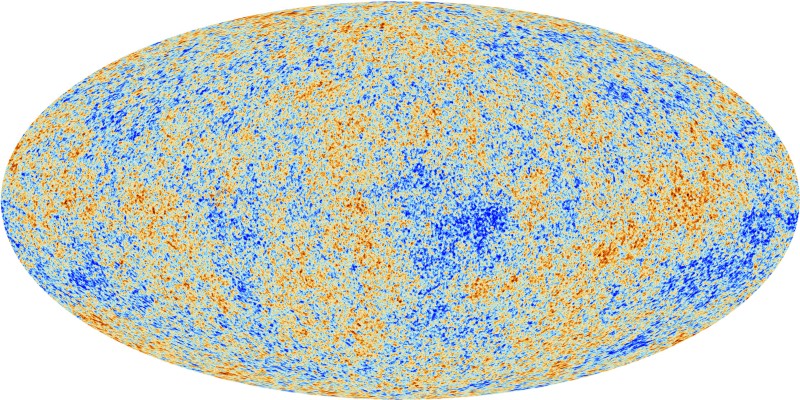
In parallel, the space expands, which increases the wavelength of the photons, because of which they lose energy. As a result, the photons lose enough energy so that the electrons can be combined with the nuclei, Thomson scattering stops, and the light can propagate freely. This moment is called recombination, and the place where the photons come from is called the last scattering surface. Egg-like plots of the CMBI show hot and cold points of photons on the surface of the last scattering throughout the sky, distributed according to the conditions preceding the recombination of the Universe.
But the temperature distribution is just a piece of information encrypted in the physics of the universe of that time. In addition, the light waves contain a preferred orientation in different parts of the sky, that is, depending on the direction to the source, the light wave oscillates in one direction more than in the other. This orientation β the preferred direction of oscillation of the wave β is polarization.
Polarization
Polarization is easier to imagine than temperature. Polarization of photons CMPI and the surface of the last scattering are the fruits of Thomson scattering, and not a complex mixture of scattering and oscillation, which occurs due to falling into regions with high density of dark matter and outside photon pressure, as is the case with temperature. In other words, despite the prevalence in the Universe, dark matter does not affect the polarization of CMPI photons. Polarization can also occur due to gravitational lensing, and dark matter physics and galactic clusters participate in this process. But in the article I consider only the polarization on the surface of the last scattering.

To understand how Thomson scattering leads to polarization, you need to understand how this process occurs. His simple explanation is a collision of two objects, and, as for almost any physical concept, a simple explanation will be incomplete. To clarify, we need to understand three things:
1. photons consist of electric and magnetic fields,
2. electrons begin to move, having fallen under the action of an electric field,
3. during acceleration, the electron emits a photon, and most often at an angle of 90 degrees to the direction of motion.
In the context of our theme, a photon from CMDF is absorbed by the electron, and the electron is accelerated in the direction of the electric field of the photon. As a result, the electron emits a new photon so that its electric field is directed in a certain direction, and has the same frequency as the original photon. This is what gives polarized light: a photon from a region where, on average, the photon electric field is oriented in a certain direction.


But this is not enough to polarize KMFI. We also need a special configuration of electrons and photons, when an electron "sees" hot photons from above and below, and colder ones - from left and right. This arrangement, the hot spots opposite each other, and the cold opposite each other, is known as the
quadrupole .

With the existence of a quadrupole arrangement around the electron, the incoming photons from the hot sections accelerate the electrons more strongly than the cooler photons. The light emitted by the electron becomes polarized, since most of the electric field strength will be aligned with the hot spots. It also turns out that only the quadrupole leads to polarization β more complex configurations of hot and cold areas do not lead to the observed polarization in the CMBR.


So, repeat.
β’ Photons consist of electric and magnetic fields, and accelerate an electron during interaction.
β’ Due to acceleration, the electron emits a new photon.
β’ Quadrupoles, visible by electrons, accelerate an electron in such a way that photons re-emitted by it are polarized.
β’ Finally, only quadrupoles lead to observable polarization in the CMBR.
Configure the quadrupoles
So, for the emergence of polarization, we need quadrupoles. How to get them? There are two main mechanisms for their production: density fluctuations and gravitational waves.
Density fluctuations lead to the observed temperature distribution. There are dense regions of crumpled dark matter (and, to a slightly lesser extent, ordinary matter), attracting photons and electrons. In the first part, we have already described how this works and leads to the creation of hot and cold areas. So, where the temperature fluctuations, there must be fluctuations of polarization.
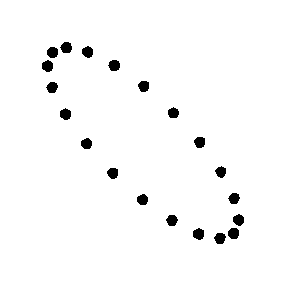
Image of particle ring deformation during the passage of a gravitational wave. In KMFI, stretching makes photons colder and compression makes hotter, which creates quadrupoles leading to polarizationGravitational waves create quadrupoles differently, stretching and compressing space. The pictures above show how the ring of particles will change with a passing gravitational wave. These deformations also affect the wavelength, causing the photon to look hotter if it is in a shrinking region, and colder in a stretching region. From the pictures it is easy to understand how hot areas appear above and below the electron, and the cold ones - on the left and on the right.

What about B-mod?


A special type of polarization, B-mode, has recently been widely reported in the press. How are they related to the described polarization?
Each polarization field can be divided into two parts: the part in which particles emanate from a certain point in the center (E-mode), and the part where particles spin to the right or left around a certain point (B-mode). If we recall the institute course in physics, then the first case corresponds to radiation without turbulence, and the second to radiation without divergence. The names E- and B- come from the analogs of the fields appearing in Maxwell's equations in vacuum, where the field E has no twist, and the field B has no divergence.
Density fluctuations β when we have a quadrupole distribution of hot and cold regions around an electron β work on E-mode radiation, and gravitational waves β stretching rings β lead to the appearance of both E-modes and B-modes. As a result, B-modes in polarization of the CMBI are produced only by gravitational waves (if we are talking exclusively about the surface of the last scattering), and E-modes appear both as a result of gravitational waves and density fluctuations. Since density fluctuations affect much more strongly than gravitational waves, the E-mode signal should dominate density effects, which coincides with observations. Therefore, the measurement of B-modes is the main goal of experimenters seeking to see prehistoric gravitational waves at KMFI.
Therefore, the search for B-mode is a priority for the cosmological community. A little earlier, the BICEP2 team announced the discovery of prehistoric B-modes, but this analysis was questioned and it requires additional observations. Several experiments are being prepared, from Planck to EBEX, SPTPol, Spider and others.
Of course, we will wait for a large amount of news telling about these experiments. And shedding light on the nature of the early Universe, we may even be able to detect the most elusive trace in the residual glow of the Big Bang: ripples on the very fabric of space!